Reports: ND1052907-ND10: Large 'Molecular Panel' Based Metal-Organic Architectures for Gas Storage and Catalysis
Gellert Mezei, PhD, Western Michigan University
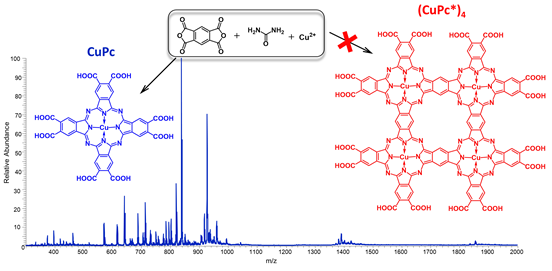
Figure
Figure 3. Electrospray ionization mass spectrum of tetrakis-(N-(4-pyridyl)phthalimide) copper phthalocyanine, showing the molecular peak (M) as well as fragments resulting from the successive loss of the terminal groups (X).
Figure 4. Absorption features suggest aggregation of coumarin-decorated copper phthalocyanine. Fluorescence quenching is assigned to the aggregation-induced energy transfer between chromophores, as observed from ultrafast fluorescence and anisotropy decay.
Figure 7. Oxidation of di-n-butyl sulfide with H2O2 monitored by 1H NMR spectroscopy in CD3OD. The catalyzed reaction (0.5 mol% CuPc) is complete in ~ 5 hours, while the uncatalyzed reaction is still incomplete (90%) after 13 hours. No di-n-butylsulfone byproduct is detected in either case.