Reports: ND554684-ND5: Spectro-Electrochemical Microscopy of Single Nanoparticle Electrocatalysts
Christy F. Landes, PhD, Rice University
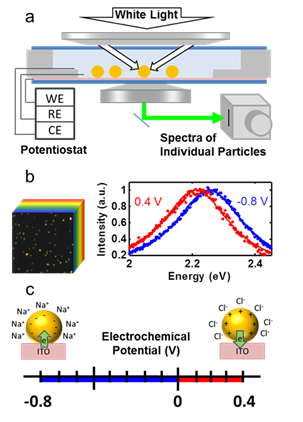
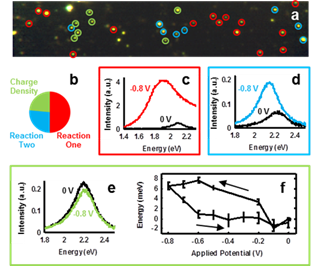
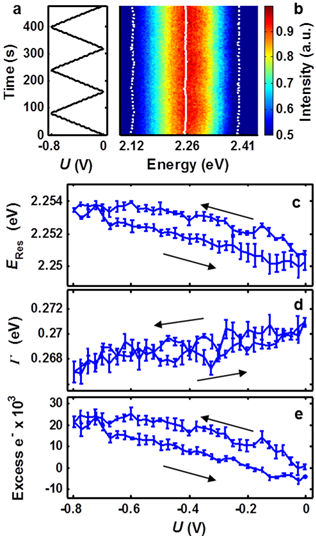
Christy F. Landes, PhD, Rice University
Reports in the ACS PRF Annual Report are published as submitted by the Principal Investigator.
Copyright © 2025 American Chemical Society