Reports: ND1052907-ND10: Large 'Molecular Panel' Based Metal-Organic Architectures for Gas Storage and Catalysis
Gellert Mezei, PhD, Western Michigan University
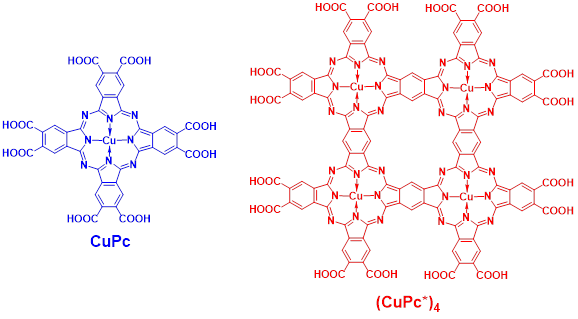
Figure 1. Structures of monomeric phthalocyanine octacarboxylic acid
CuPc and cyclic tetrameric phthalocyanine hexadecacarboxylic acid (CuPc*)4.
1. Tetrakis-(N-((4-carboxy)phenyl)phthalimide) copper phthalocyanine
2. Tetrakis-(N-((4-ethoxycarboxy)phenyl)phthalimide) copper phthalocyanine
3. Tetrakis-(N-((3,5-dicarboxy)phenyl)phthalimide) copper phthalocyanine
4. Tetrakis-(N-(4-pyridyl)phthalimide) copper chthalocyanine
5. Tetrakis-(N-(p-tolyl)phthalimide) copper phthalocyanine
6. Tetrakis-(N-(pyrazole-4-yl)phthalimide) copper phthalocyanine
7. Tetrakis-(N-(anthracene-2-yl)phthalimide) copper phthalocyanine
8. Tetrakis-(N-(pyrene-1-yl)phthalimide) copper phthalocyanine
9.
Tetrakis-(N-(4-(trifluoromethyl)-2H-chromene-2-one-7-yl)phthalimide) copper
phthalocyanine
Figure 3. Electrospray ionization mass spectrum of tetrakis-(N-(pyrazole-4-yl)phthalimide) copper phthalocyanine.
Figure 4. UV-vis spectra of variously
substituted copper phthalocyanine building blocks.
Figure 5. GC-MS analysis of
the reaction mixture obtained by the uncatalyzed (bottom) and 0.5 mol%
CuPc-catalyzed (top) oxidation of dibutylsulfide (at 3.25) to dibutylsulfoxide (at
5.41) by hydrogen peroxide after 2 hours.