Back to Table of Contents
46040-AC10
Spectroscopy of Photovoltaic and Photoconductive Doped-Oxide Electrodes Related to Photocatalysis and Solar Energy Conversion
Daniel R. Gamelin, University of Washington
We have, with
ACS-PRF support, been studying the mechanisms of sensitized photoinduced charge
separation in transition-metal-doped oxides by photocurrent, absorption, and
magnetic circular dichroism (MCD) spectroscopies. Our experiments allowed us to
directly observe the electronic transitions responsible for initiating charge
separation and we found a strong correlation between photocurrent activity and
charge transfer transitions between the transition metal dopant and
semiconductor. Similar to Co2+: ZnO,1 we demonstrated
that sub-bandgap transitions can give rise to photocurrent by charge transfer
and excitonic state mixing. In the literature it has been suggested that d-d
transitions are responsible for sub-bandgap photocurrent.2,3 Our
results indicate that ligand field transitions are not directly photoactive but
may enhance photoelectrochemical responses by sensitization of charge transfer
transitions.
Synthesis and construction of photovoltaic cells. Films of Co2+:ZnO,
Ni2+:ZnO, Cr3+:TiO2, Co2+:TiO2,
and Ni2+:InTaO4 were fabricated using a variety of
techniques including spin coating of nanoparticle colloidal suspensions,4,5
sol solutions, and products of solid state synthesis6 on transparent
conducting glass. Photovoltaic cells were constructed from the oxide
photoanodes, an I-/ I3- electrolyte,
and platinum electrode. Many of the cells responded strongly to light, with
current changes on the order of micro‐amps upon illumination with a 150 W halogen lamp.
Spectroscopy and photocurrent activity of transition
metal doped oxides.
Electronic transitions of Co2+:ZnO, Ni2+:ZnO, Cr3+:TiO2
and Co2+:TiO2 have been well studied by our group through
absorption and MCD spectroscopies.1,4,5,7 Building on our
fundamental understanding of these materials and using ligand field and charge
transfer calculations, we were able to give detailed electronic assignments to
our spectra (Figure 1). As for Ni2+:InTaO4, a sol-gel
synthesis of Ni2+:InTaO4 was successfully developed, as
confirmed by XRD, and electronic absorption and MCD spectra of the material
were collected for the first time (Figure 2). We observed a bandgap of 3.8 eV
in InTaO4, which narrowed to 3.2 eV upon nickel doping and the rise
of a charge transfer transition. Pairing photocurrent action spectroscopy with
the above experiments, we consistently observed internal quantum efficiencies
(IQEs) corresponding to bandgap, metal-to-ligand conduction band charge
transfer (MLCBCT), ligand valence band-to-metal charge transfer (LVBMCT)
and some ligand field transitions (Figures 1 and 2). As a result, we successfully
identified the electronic transitions giving rise to photocurrent.
Photoinduced charge separation processes. Several general
trends were observed in our photocurrent experiments. Direct bandgap excitation
always gave the highest efficiencies, followed by charge transfer, and certain
spin-allowed ligand field transitions. The d‐d transitions that were inactive appear to be much
lower in energy than the charge transfer transitions. A list of photoactive
transitions in the metal oxides studied is given in Table 1. These trends along
with a comparison of IQE values and transition energies, suggest that photoinduced
charge separation in sub-bandgap regions are facilitated by the degree of
mixing between MLCBCT or LVBMCT and excitonic states. An
example of the energy level diagram leading to charge separation for Co2+:TiO2
is given in Figure 3. These results agree quite well with the proposed
configuration interaction model in Figure 4, where escape of the photogenerated
carrier is governed by mixing between charge transfer and excitonic states. If
ΔE is small, the large effective Bohr radius will allow extensive mixing
of the two states and carrier migration is possible (Figure 4a). On the other
hand, if ΔE is large, the effective Bohr radius will be small, limiting
the amount of mixing of the two states. As a result, carrier migration to the
electrical contact will be inefficient (Figure 4b). As for the ligand field
regions, IQEs were generally extremely small and the above method did not
apply. This suggests that they are not directly photoactive, but may improve
photoresponses by sensitization of charge transfer transitions.
Further studies. Experiments in synthesizing Ni2+:InTaO4
will continue for electrochemical studies and determination of band potentials.
Photoconductivity experiments are in progress. As new oxide materials are being
prepared in our lab, these results will be used to understand the role of
doping in carrier generation, where the charges can be used for driving uphill
redox reactions, such as water splitting.
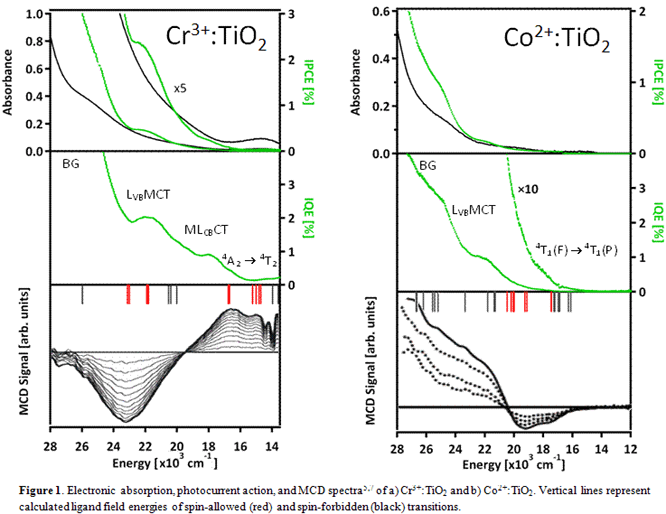
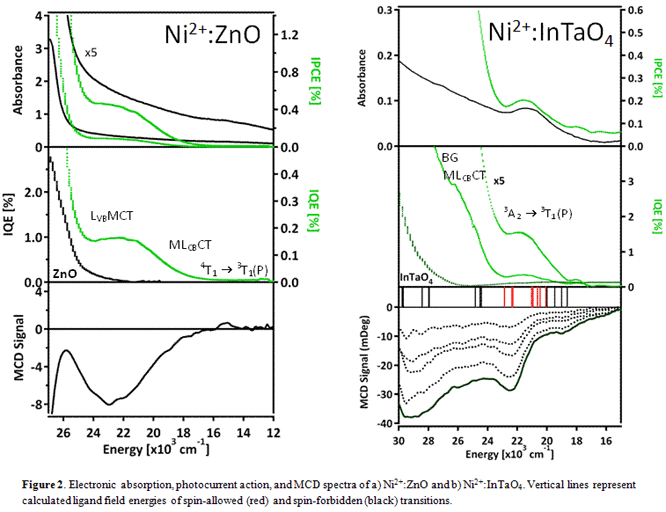
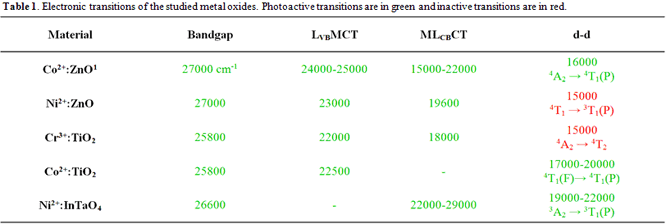
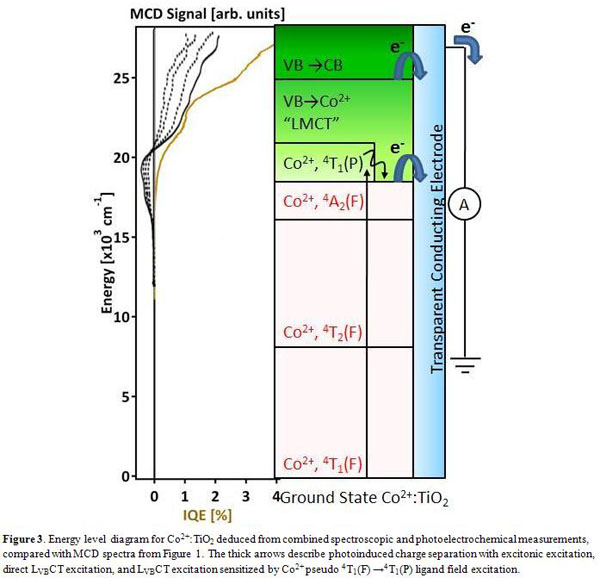
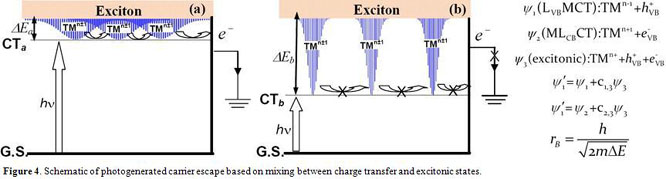
References
1.
Liu,
W. K., Mackay Salley, G., Gamelin, D. R. J.
Phys. Chem. B. 2005, 109,
11486-11495.
2.
Kato,
H., Kudo, A. J. Phys. Chem. B. 2002, 106, 5029-5034.
3.
Zou,
Z., Ye, J., Kazuhlro, S., Arakawa, H. Nature. 2001, 414, 625-627.
4.
Schwartz,
D., Norberg, N. S., Nguyen, Q. P., Parker, J. M., Gamelin, D. R. J. Am. Chem. Soc. 2003, 125, 13205-13218.
5.
Bryan,
J. D., Santangelo, S. A., Keveren, S. C., Gamelin, D. R. J. Am. Chem. Soc. 2005,
127, 15568-15574.
6.
Zou,
Z., Ye, J., Kazuhlro, S., Arakawa, H. J. Mater. Res. 2002,
17, 1419-1424.
7.
Bryan,
J. D., Heald, S. M.,Chambers, S. A., Gamelin, D. R. J. Am. Chem. Soc. 2004,
126, 11640-11647.
Back to top