Back to Table of Contents
47110-G10
Control of Methane Hydrate Formation at the Molecular Level
Tadanori Koga, State University of New York at Stony Brook
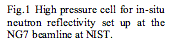
Natural gas hydrates owe their existence
to the ability of water molecules to assemble via hydrogen bonding and form
polyhedral cavities in which trapped methane molecules reside[1,2]. Though an
initial interest in understanding gas hydrate formation focused on flow
assurance to avoid gas pipeline plugging, it is now being considered for
applications such as an alternative to desalination technology[3], potentially
a huge natural gas reserve[2], and natural gas transport alternative to
liquefied natural gas due to high energy density (160 cubic meters of natural
gas per cubic meter of gas hydrate at STP)[4]. A recognizable problem in
utilizing the versatility of gas hydrate routes is the uncertainty in the
hydrate formation process that can take from few minutes to several days. In
this proposed research, we aim to understand the mechanism of the formation at
the molecular scale, which have not been elucidated yet.
As a model system,
we are focusing on a stationary interface separating
methane (hydrophobic) and liquid water where the hydrate formation develops.
The experimental technique used is neutron reflectivity (NR), which is
sensitive to interfacial structures under compressed gases. At the same
time, laser light scattering (LS) is also utilized as a complementary technique
to study the growth of the hydrate crystals at the micron-scale. In order to mimic environmental
conditions (0<T<10�C
and 5<P<15MPa) for laboratory-based experiments, we have built a high-pressure
cell made of stainless steel (Fig.1).
In-situ
NR experiments using the high-pressure cell were carried out at the NG7
beamline of the National Institute of Standards and Technology (Gaithersburg,
MD). Deuterated methane (CD4) and deuterated oxide (D2O)
were used for NR due to low absorption for neutrons and a scattering contrast. A
two-dimensional detector was utilized to characterize the layer structures
normal to the surface (specular components) as well as lateral surface
structures (diffuse components) simultaneously. Fig. 2 shows the representative
scattering profiles from a D2O/CD4 system at T=5.5�C. We found the drastic increase
in the diffuse scattering occurred at P>Pc (the critical pressure
of 3.8 MPa) within 10 min exposure (Fig. 2b) compared to those at P<Pc
where no time evolution of the scattering profile was observed for 10 h (Fig.
2a). The change indicates the existence of the rough surface at the nanometer scale due to the formation of methane
hydrate at the interface. In addition, the nucleation times for the surface hydrate
formation were estimated to be about 10 min and remained unchanged regardless
of the pressures used for the experiments (Fig.3).
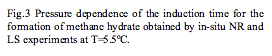
Further,
we studied the formation of the hydrate structures at the micron-scale by using
LS. For LS experiments, the reflected beam from the D2O/CD4
interface was monitored by using a photosensitive detector as a function of time. It was found that
the formation of the micron-scale surface structures caused a sharp
discontinuity in the intensity, leading to the induction time at the given
environments. As summarized in Fig.3, the induction time determined by the LS
experiments increased up to several days as approaching the critical pressure,
which can be explained by the classical nucleation theory and has been previously
observed in bulk methane hydrate experiments. Thus,
this is the first time, to the best of our knowledge, to demonstrate
experimentally that the nanometer-scale methane hydrate crystals can be
formed rapidly even near the phase boundary. We are currently studying the effect of surfactants, which
are known to accelerate the formation of the macroscopic methane hydrate
crystals and could control the growth of the nanometer-scale hydrate crystals
as well.
References
1. Sloan, E.D.,
1998. Clathrate Hydrates of Natural gases, 2nd Ed., Marcel Dekker,
New York.
2. "Gas Hydrates
and Clathrates", 2007. J. Pet. Sci.& Eng...56(1-3), D. Mahajan and C. Taylor, Eds.
3. Osegovic,
J.P., Tatro, S.R., Holman, S.A., Ames, A.L., Max, M.D. J. Pet. Sci.& Eng.
56(1-3), 42-6.
4. Gudmundsson,
J.S., Borrehaug, A., 1996. Frozen hydrate for transport of natural gas. Proc.
of 2nd Int. Conf. Natural Gas Hydrates, 415-22.