Back to Table of Contents
44403-G5
Synthesis of Inorganic Electrodes with Controlled Interfacial Structures by Crystal Engineering in Electrodeposition
Kyoung-Shin Choi, Purdue University
The overall goal of our research is to produce various inorganic polycrystalline electrodes with optimum interfacial structures that can enhance desired functional properties. We achieve this goal by precisely tuning the individual shapes of particles composing polycrystalline electrodes and studying their morphological effects on physical and chemical properties. . Electrodeposition is used as the main synthetic method as it can produce a variety of materials as thin film-type electrodes. The most significant achievements made during the first budget year are summarized below.
(1) Growth of branched Cu2O crystals
We have identified conditions to grow Cu2O crystals using two different branching mechanisms: overpotential-limited branching and diffusion-limited branching. Overpotential-limited branching growth occurs in overpotential regions lower than those enabling faceting growth, whereas diffusion limited branching growth occurs at overpotential regions higher than those enabling faceting growth (Figure 1).
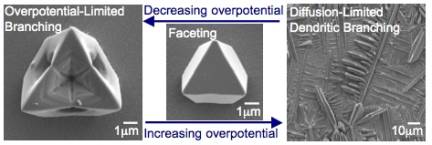
Figure 1. Comparison of the overpotetial-limited branching and the diffusion-limited branching.
Overpotential-limited branching is caused by the formation of a concentration gradient around a growing crystal during deposition. The presence of this concentration gradient also creates a reduction potential gradient, and therefore an overpotential gradient across the crystal (the central part of the face will experience a lower overpotential than the corners protruding into the more concentrated regions). Branching growth occurs if the chosen deposition potential has the minimum overpotential necessary to reduce [Cu2+] ions in the bulk solution. When the concentration gradient forms, the central part of the face will not have sufficient overpotential to continue growth, whereas the protruding regions of the crystal will continue to grow, thereby resulting in branching growth (Figure 2a). When the overpotential increases, the region in a crystal that has enough overpotential to grow can expand toward the center of the facet decreasing the degree of branching (Figure 2b-c). Therefore, the degree of branching in overpotential-limited branching systematically decreases as the overpotential increases (Figure 3). This new branching mechanism allows for systematically tailoring crystal shapes by simply changing deposition potentials. This ability will have a direct impact on enhancing and tuning catalytic properties of Cu2O.
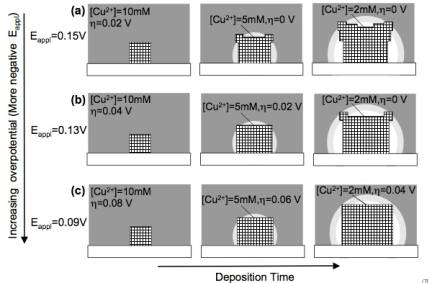
Figure 2. Schematic representation showing the evolution of overpotential-limited branching.

Figure 3. Morphology diagram of Cu2O branching growth caused by the overpotential-limited branching. For cathodic deposition, a more positive applied potential creates a less overpotential.
Diffusion-limited branching growth can be stabilized when the deposition rate exceeds the diffusion rate (Figure 1). However, stabilizing diffusion-limited branching of Cu2O is not straightforward because increasing the potential/current necessary to create dendritic Cu2O results in the deposition of Cu metal instead. Even if the initial applied potential was chosen to produce pure Cu2O, the pH drop accompanied by fast production of Cu2O triggered the deposition of Cu metal during Cu2O deposition (the formation of Cu is more feasible at lower pH). To overcome this problem, we utilized a buffered plating solution and obtained dendritic growth of pure Cu2O. The dendritic branching growth provides a mean to create high surface area electrodes with significantly enhance reactivities. Our preliminary results show that the dendritic Cu2O films exhibit superior photoelectrochemical properties to those composed of faceted Cu2O crystals.
(2) Synthesis of Polycrystalline Sn and SnO2 Films with Wire Morphologies
Among various polycrystalline morphologies, the wire morphology is of strategic importance for photoelectrochemical applications, because its one-dimensional architecture can minimize the randomness of the charge carriers' pathway and achieve superior charge transport properties when compared to other polycrystalline morphologies of equal surface areas. We have identified conditions to produce Sn and SnO2 films with wire morphologies. Sn films with wire morphology were produced by cathodic deposition using a DMSO medium. Both the aging of the plating solution and passing electric charge through the cell prior to deposition were critical for obtaining wire morphologies. These processes affected the composition of Sn species in solution, which drastically altered the morphologies of Sn deposits from cauliflower shape to wires (Figure 4a-b). Introduction of gas bubbling (e.g. N2, O2) during electrodeposition resulted in more uniform growth of wires (Figure 4c).
The resulting Sn films can be converted to SnO2 films by thermal oxidation while preserving their original morphologies. The SnO2 films with wire morphology exhibited superior photoelectrochemical performance compared to cauliflower morphology, indicating that fine tuning of wire growth may lead to the construction of highly efficient and cost-effective electrodes for photoelectrochemical applications (Figure 4d-e). We will further investigate whether the wire morphology is also advantageous for using Sn and SnO2 electrodes as anodes for Li-ion batteries.
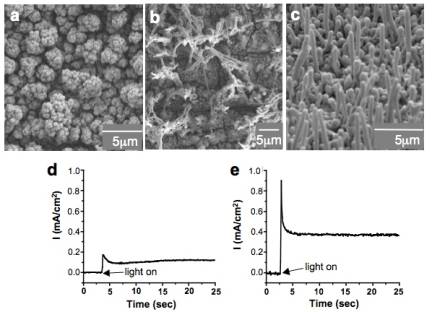
Figure 4. SEM images of Sn films (a) with cauliflower and (b-c) with wire morphologies. Photocurrent measurement of samples a and c after thermal oxidation are shown in (d) and (e), respectively.
Back to top